An Institute-Strategic Programme at the Department of Mathematics and Physics, Faculty of Science and Technology, University of Stavanger.
SPACT is a founding member of the national PACT network NPACT. Started in 2017, it has grown from 27 to now (2025) 44 senior and junior scientists at 8 academic institutions in Norway + CERN.
SPACT is the largest node of NPACT, and has throughout been coordinating the activities of the network.
Our researchers
Research highlights
Gravitational waves and LIGO
There are expected to be around 100 million black holes in our galaxy.
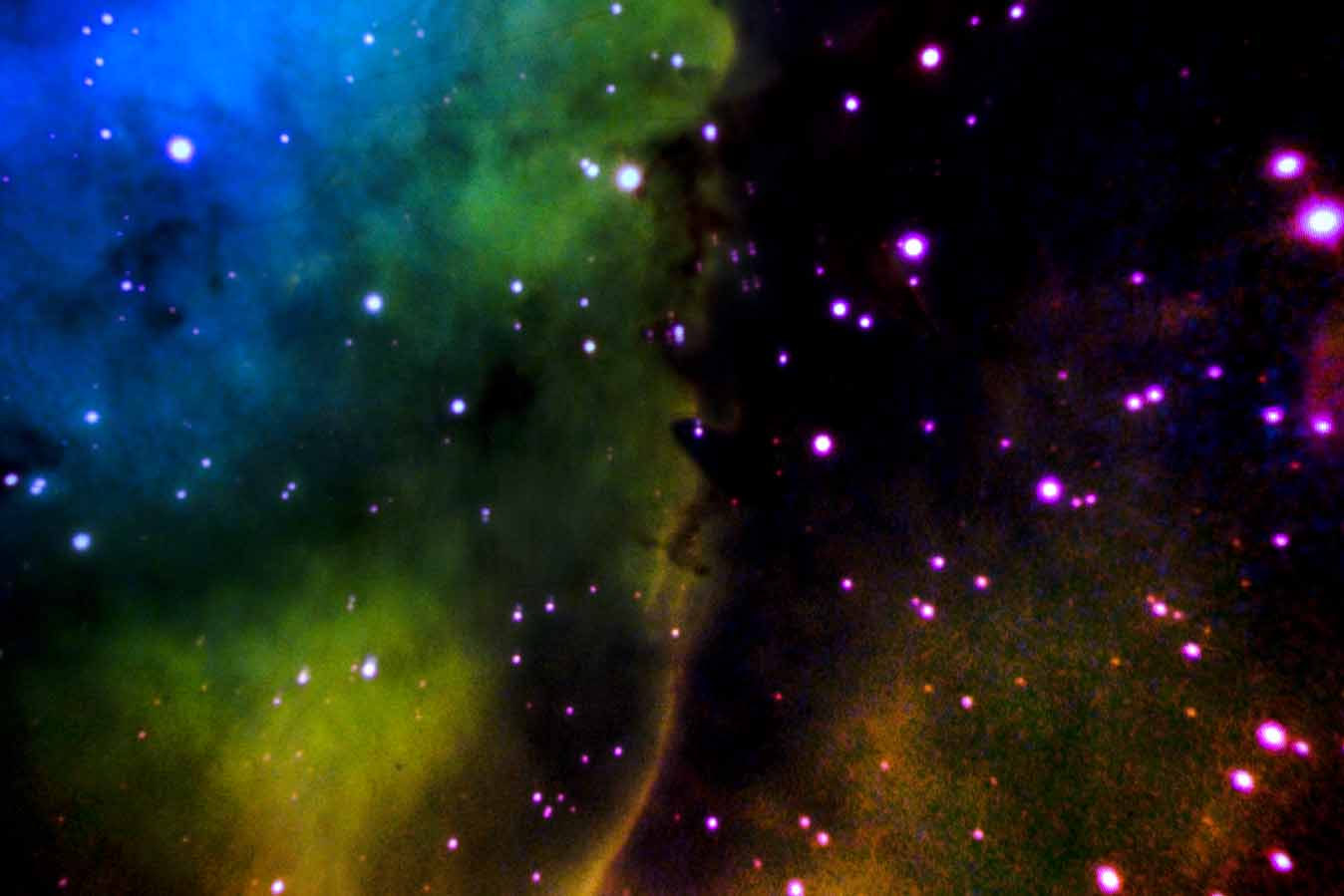
Most of these black holes formed from large stars that ran out of nuclear fuel and collapsed in on themselves under the force of gravity. These black holes are difficult to detect, because their gravitational field is so strong, they trap even light from escaping. Some of these black holes have companion stars and we can infer the existence of the black hole from the behaviour of its companion star, which we can see. But what about all the other black holes?
Einstein’s theory of gravity predicts how black holes should behave, even if we cannot see them. One of the predictions of Einstein’s theory is that black holes orbiting each other should emit gravitational waves. Gravitational waves are like earthquakes in space. Black holes are so massive and orbit each other so closely and so quickly, that they make the entire universe shake. This shaking travels through space as gravitational waves and can reach the Earth. By the time they reach Earth they are expected to be very weak and barely noticeable.
In the past fifty years, a huge experimental effort has been made to detect these gravitational waves. This effort was finally successful in 2015 with the Advanced LIGO project. The LIGO project required building the most sensitive machines ever built, using lasers to measure the tiny movements of large mirrors, that are kilometers apart. Using this technology, LIGO was finally able to detect the gravitational waves from two black holes, orbiting each other and finally crashing together. This discovery wowed the world and was awarded the Nobel Prize for Physics in 2017.
Einstein’s theory of gravity does a very good at predicting how black holes behave and the gravitational waves emitted. But Einstein’s theory might not be entirely correct. There are several reasons why scientists think the theory might not be complete. At the largest scales, Einstein’s theory predicts the Universe should not be expanding as fast as it is observed to be expanding. It also predicts that galaxies should not be able to hold themselves together using just the material we can see and they should in fact be flying apart. Scientists have tried to explain the expansion of the Universe and the behaviour of galaxies by invoking Dark Energy and Dark Matter, but it is not known what these things are. Einstein’s theory predicts that around 95% of the total energy of the Universe is in an unknown form.
At the smallest scales, quantum theory predicts that empty space should be filled with large amounts of energy, much larger amounts than needed to explain the expansion of the Universe. Einstein’s theory of gravity predicts that this vacuum energy is so large, it should prevent the Universe from existing. Clearly the Universe exists and it is not known why these predictions are so wrong, or which new theory can correctly explain how we exist. In addition, Einstein’s theory is mathematically inconsistent with quantum theory. Both theories work very well to explain observations in each their own domain, but the two theories fail when we try to use them together.
One place where this failure is most relevant is for black holes. It has been proven mathematically that some aspect of our understanding about black holes must be wrong somewhere. This mathematical proof was recognized with the Nobel Prize for Physics in 2020. But the proof does not tell us what is wrong, or how to correct it. By observing very closely how black holes behave in nature, we can try to spot what is going wrong. This is the work being carried out at the University of Stavanger.
Since LIGO’s first discovery in 2015, detector technology has improved. As of 2024 we can detect multiple black hole collisions every week. This has given astronomers a new tool to explore the Universe and observe things that cannot be seen with traditional telescopes. In particular, we can check the detailed predictions of many black hole collisions, looking for hints of what is going wrong and where. All of this was completely impossible before gravitational wave detectors.
Another exciting event occurred in 2017, when two colliding neutron stars were observed using gravitational waves. Unlike black holes, neutron stars contain matter that can be seen by ordinary telescopes. When these two neutron stars collided, a powerful jet of gamma rays was produced and heavy nuclei were flung out into space, similar to the heavy elements we have on Earth like gold and platinum. This event was so unique that a third of all living astronomers worked on the observations, making it one of the most studied events in the history of astronomy.
A further advance came in 2023, when a new technique was used to detect gravitational waves. Instead of detecting signals here on Earth, astronomers used disturbances in the radio signals coming from rotating neutron stars, to infer gravitational waves passing through the galaxy. Unlike the LIGO gravitational waves, these PTA signals appear to come continuously from all directions in the sky. While it is likely that orbiting black holes contribute to this signal, early indications are that some of the signal may originate from much earlier in the Universe’s history, giving observational access to high energy processes that are impossible to recreate here on Earth.
The field of gravitational waves is still very young. Multiple major advances have happened in the past few years, but new experimental techniques are being developed all the time, along with new ideas of what might be discovered. There are plans for the next generation of gravitational wave detectors to be built in Europe and the United States and advanced plans from the European Space Agency to place a gravitational wave detector into space in the next decade. This LISA detector will have arms that are not kilometers long, but millions of kilometers, giving observational access to entirely new classes of objects. Already, gravitational wave astronomy has provided surprises, overturning existing ideas of what is out there in our Universe. It is not known what the new discoveries will be in the coming decades, but it is an exciting time of discovery.
Links:
https://ligo.org/ https://journals.aps.org/prl/abstract/10.1103/PhysRevLett.116.061102 https://iopscience.iop.org/article/10.3847/2041-8213/acdac6 https://www.nobelprize.org/prizes/physics/2017/summary/ https://www.nobelprize.org/prizes/physics/2020/summary/
Gravitational waves and Dark Matter
Visible objects in our Universe like stars, planets or human beings are composed of atoms that, in turn, are made of protons, neutrons, and electrons.
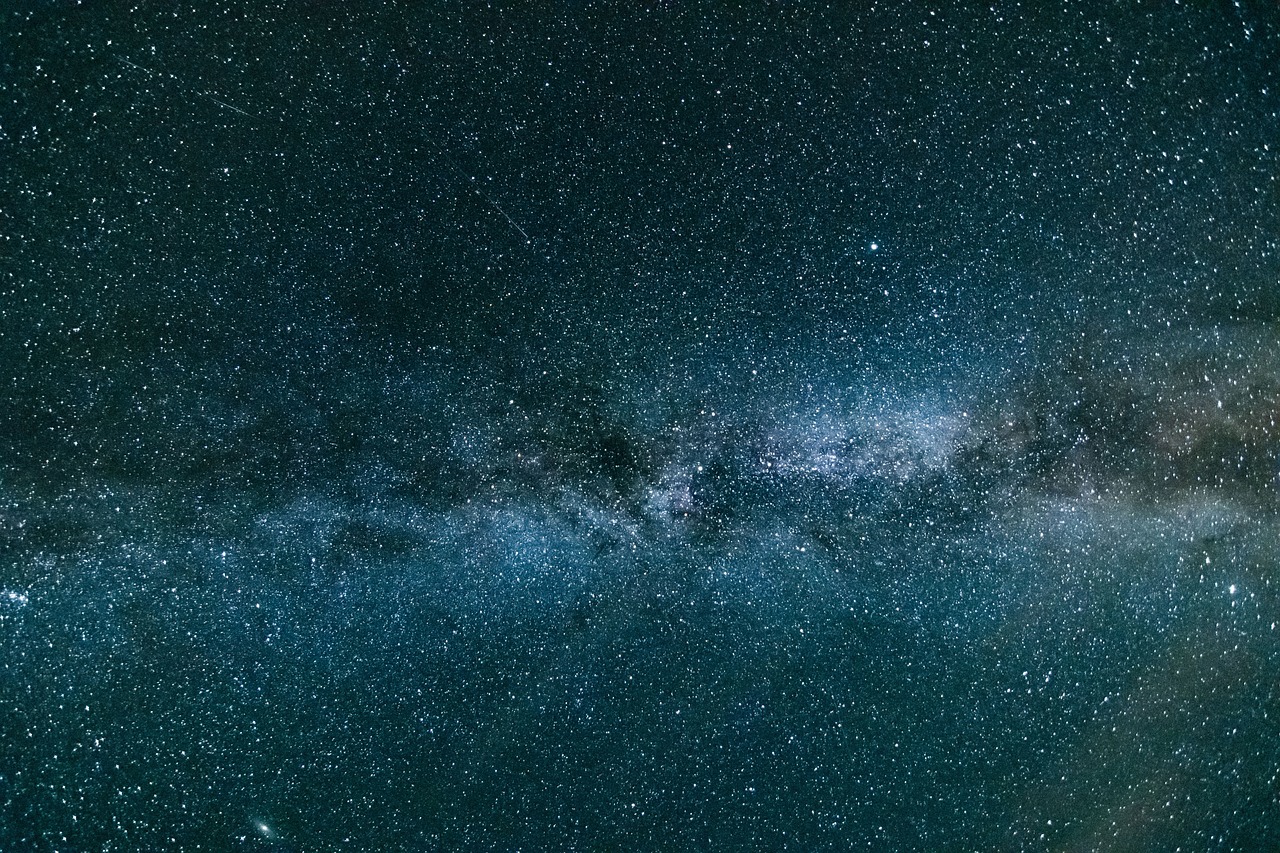
But only about 16% of the matter in our Universe is composed of these particles, the nature of the remaining 84% is unknown and this component is usually referred to as "dark matter". Is this mysterious matter formed by new particles? And if so, how could we detect them? These are questions that Helena Kolesova and Daniil Krichevskiy are trying to answer.
But first of all, let us explain why scientists believe that dark matter exists in the first place. Since 1960’s, astronomers were able to “weigh” the matter in galaxies by observing their spinning: the more massive the galaxy is, the quicker the stars rotate around the galaxy center. To their surprise, the mass of the galaxies measured in this way was about 5-times larger than what was expected based on counting the stars and other visible matter. Similar discrepancy was observed for galaxy clusters and thanks to further modern astronomical observations, there is a strong evidence for presence of invisible matter in our Universe.
Also our home galaxy, the Milky Way, is believed to be surrounded by a spherical dark matter halo (see image?). Consequently, dark matter should be passing through Earth as the latter is racing through our galaxy. Physicist have built different types of detectors and observed carefully every atom inside it for several years, however, no events of a dark matter particle striking an atom in such detectors was reported up to now. This means that dark matter interacts yet more weakly with ordinary matter or is much lighter than we thought and we need new experimental techniques to reveal it. One of our research goals is to find ways how to probe dark matter particles if they were lighter than ordinary protons and, hence, were not able to strike the atoms in the existing detectors strongly enough to be observed.
Unfortunately, it is also possible that dark matter interacts with ordinary matter so weakly that we will be never able to detect it on Earth. On the other hand, dark matter definitely interacts through gravity. Consequently, newly established gravitational wave observatories could also help to shed light on dark matter. In particular, if the dark matter particles went through a violent process of a "phase transition" in the early Universe, this could have left an inprint as a so-called "gravitational-wave background". This signal could be potentially detectable by the planned experiment LISA.
Effective field theory at high and low energies
The laws of physics describe nature across more than forty orders of magnitude in scale: from the most microscopic, subatomic particles to the largest known structures in the universe.
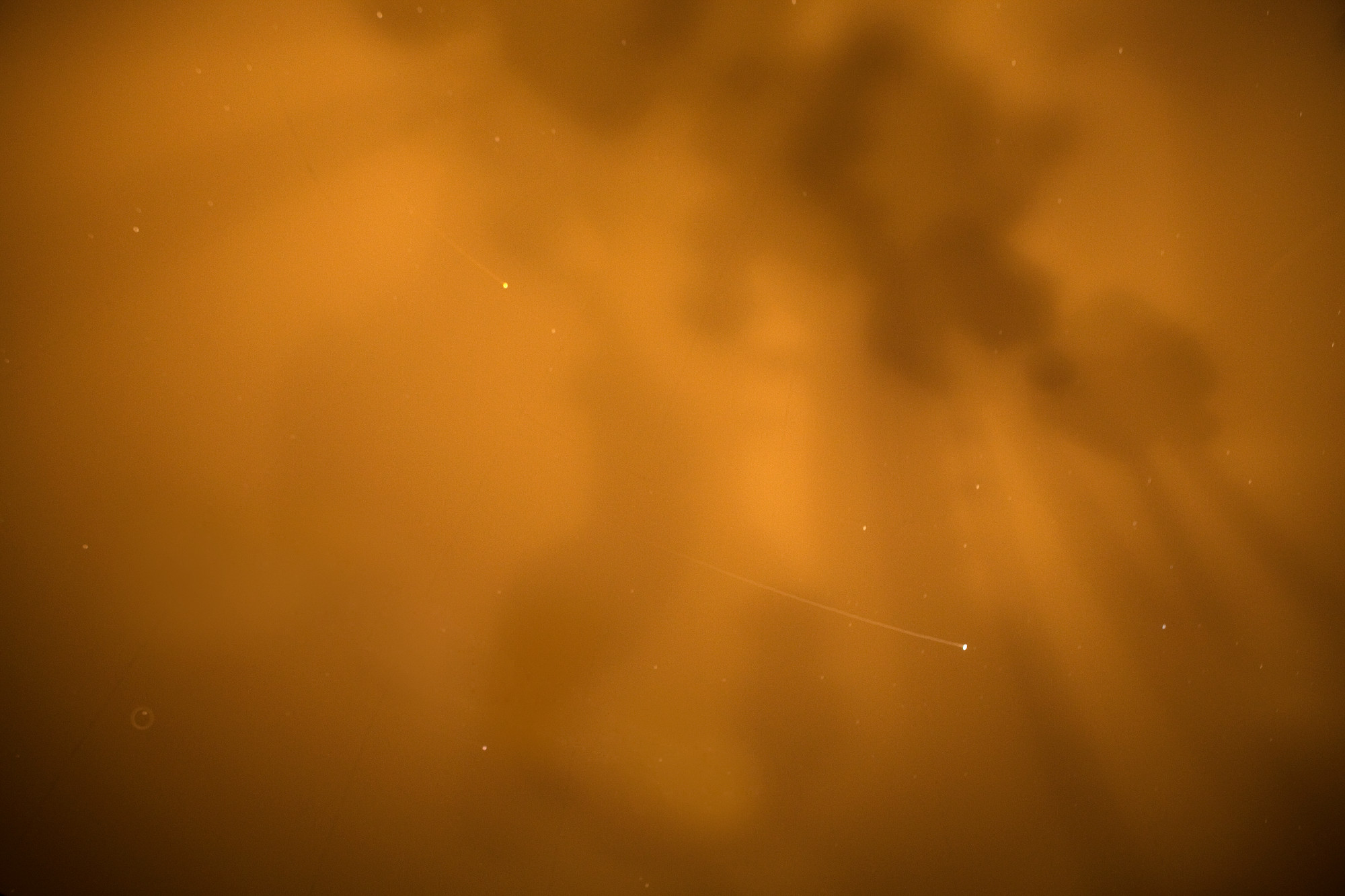
To our best understanding, the laws of nature are hierarchical. The extremely complex observed behavior of matter, often vital for everyday life – think of the variety of materials around us, both natural and engineered, or atmospheric phenomena and the planetary dynamics – emerge from simple underlying rules and principles. Ultimately, all natural phenomena boil down to the four fundamental interactions: the strong and weak nuclear force, electromagnetism, and gravity.
Fortunately, we do not need to solve the equations describing the entire Universe every time we want to make a cup of coffee or send an email to a friend. The physical phenomena observed at different scales of resolution are largely independent of each other. In fact, this separation of scales has been essential for progress in our understanding of Nature. Newton did not need to know the detailed structure and composition of planets in the Solar System to deduce Kepler’s laws from his universal law of gravity. Many macroscopic properties of ordinary solids and fluids were mapped out well before the advent of quantum mechanics, which is needed to properly account for the microscopic structure of matter. Similarly, the quantum-mechanical description of atoms and molecules was developed before the structure of atomic nuclei was understood, and before the discovery of the elementary constituents of nucleons – quarks and gluons.
In the last half-century, the idea of separation of scales has evolved into a full-fledged mathematical formalism, known as effective field theory. This concept was motivated by the study of strong nuclear interactions among particles known as hadrons. Strongly interacting physical systems are notoriously difficult to deal with, and the strong nuclear force is no exception. Surprisingly, the lightest hadrons – pions and kaons – tend to interact weakly. The first successful application of effective field theory traced this weak interaction, as well as the comparatively small mass of pions and kaons, to the symmetry of the microscopic forces among quarks.
The great power of effective field theory lies in its ability to maximally exploit the symmetry of a physical system. All the unknown microscopic details are condensed into a handful of parameters that can be determined by experiment. Once the symmetry-based design of effective field theory was understood, its applications to a vast range of problems across physics exploded. Nowadays, effective field theory techniques play an indispensable role in the mathematical description of physics at all length scales, from elementary particles to modern solid-state physics to gravitation and cosmology.
At the University of Stavanger, we use effective field theory to tackle open problems in several domains of theoretical physics. In gravitational physics, we use it to deal with the complicated nonlinear dynamics of compact binary systems. In the domain of nuclear matter under extreme conditions, we perform state-of-the-art computations of the equation of state of neutron stars. Lastly, we apply symmetry-based effective field theory to the study of collective behavior of quantum matter such as superfluids and ferromagnets.
In the last couple of decades, effective field theory has enabled extremely productive cross-fertilization between traditionally separated areas of physics. It has helped us appreciate the universality of emergent physical laws across vastly different contexts. Alongside effective field theory, the importance of symmetry for understanding complex physical phenomena has been steadily growing. Their combination will undoubtedly play a central role in the next steps of our quest to fully understand the laws of nature.
Physics of the very early universe
The properties and evolution of our universe appears to be well described by the Big Bang model (ΛCDM).
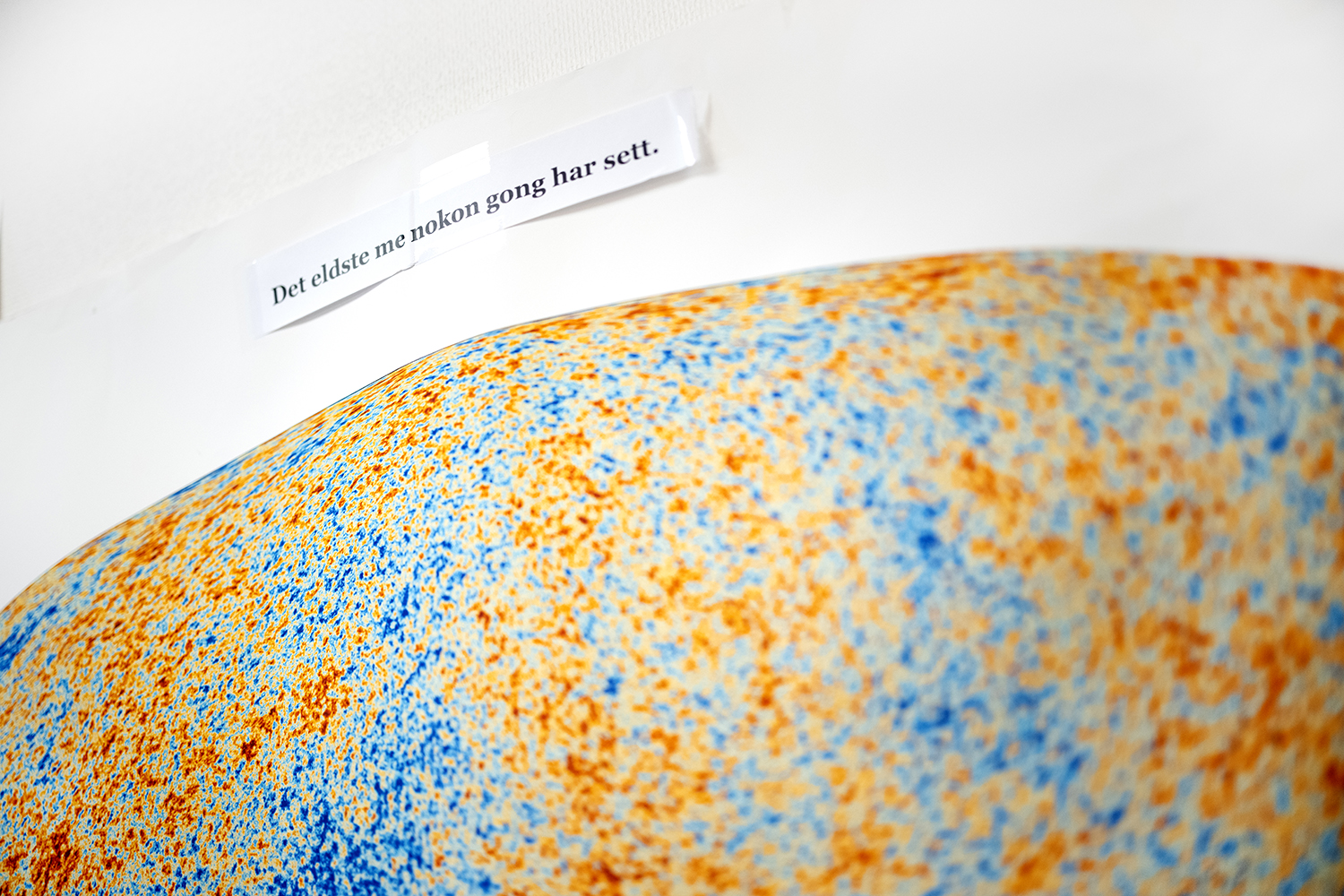
This model follows relies on general relativity with a near-homogeneous distribution of matter on large enough scales. At least 4 central issue remain to be finally resolved:
- The mechanism responsible for the observed asymmetry between matter and antimatter (Baryogenesis)
- The origin and properties of dark matter
- A proper understand of current cosmological acceleration and dark energy
- The origin of the seed for primordial structure formation
It appears that all of these phenomena belong to the realm of high-energy (quantum) field theory and fundamental particles. The current line of thought is indeed that:
- Baryogenesis is the result of out-of-equilibrium conditions near a phase transition in the early Universe
- Dark Matter is a (very) weakly interacting particle, not unlike the ones already discovered
- Dark Energy is associated with the quantum dynamics of a new field, or that there is more to gravity than General Relativity
- Primordial structure was seeded by quantum fluctuations originating in the very early Universe, enhanced by a period of accelerated expansion known as inflation
Having been under intense scrutiny for decades, all of these phenomena appear to rely on sofar undetected fields and particles. Understanding how to detect these requires sophisticated theoretical computations in the context of particle collider experiments and in the extreme conditions of the early Universe.
At the University of Stavanger, work is ongoing to use LISA to detect gravitational waves from early Universe phase transition, from primordial inflation and from Dark Matter. Gravitational waves may also be used to constrain alternative models of gravity, narrowing down options for explaining Dark Energy. The first review on the detection of phase transitions with LISA was written during the second Cosmology Working Group meeting in Stavanger (https://arxiv.org/abs/1512.06239, later updated in https://arxiv.org/abs/1910.13125).
News and events
Like tuning forks in space: A final pure tone reveals mysterious interior of neutron stars
Scientists at University of Stavanger and Goethe University Frankfurt have identified a new way to probe the interior of...
12 million for research in algebraic geometry
Professor Helge Ruddat has received NOK 12 million to investigate and classify the mathematical concept of Fano manifold...
New evidence for quark matter cores in massive neutron stars
Researchers at the University of Stavanger are now one step closer to finding out what is in the core of neutron stars. ...
In the core of a neutron star
How can the established theory of particle physics, the Standard Model, be used to predict the material properties of th...
Quark-Lab: Centre for fundamental physics research (SFF)
A proposed centre of excellence (SFF), Quark-Lab will build a bridge between the collider physics and gravitational wave...
Research prize awarded to particle physicists and cosmologists
A young an amibitious research group received the Lyse Research Prize for 2019, having been recognized on the national a...
Department of Mathematics and Physics
The Department of Mathematics and Physics performs research and offers education in the fields of Mathematics, Statistic...
Contact information
Department of Mathematics and Physics
Department of Mathematics and Physics